Features of L-amino acid adsorption on nanocrystalline anatase
Автор: Bolshakov O.I., Potemkin V.A., Grishina M.A., Galushko A.S., Merzlov S.V., Morozov R.S., Shchelokov A.O., Popov V.V.
Журнал: Вестник Южно-Уральского государственного университета. Серия: Химия @vestnik-susu-chemistry
Рубрика: Неорганическая химия
Статья в выпуске: 1 т.9, 2017 года.
Бесплатный доступ
Affinity characteristics of biomolecules, such as peptides and proteins, towards inorganic surfaces in general and to titanium oxide in particular become crucial in biotechnology and bionanotechnology. Amino acids are often used as “model” bits of peptides or proteins for studying their properties in different environments, and/or developing functional surfaces. Despite the great demand for knowledge about amino acid interactions with metal oxide surface, studies on the issue lack in consistency and represent a fragmentary picture. This paper is an attempt to study amino acid adsorption on nanocrystalline anatase systematically at uniform conditions. Features of the L-alanine adsorption have been revealed and interpreted with quantum-chemical calculations.
Titanium oxide, anatase, nanoparticles, l-amino acid, adsorption, ir-spectroscopy, nmr-spectrometry, l-аминокислоты
Короткий адрес: https://sciup.org/147160380
IDR: 147160380 | DOI: 10.14529/chem170101
Текст научной статьи Features of L-amino acid adsorption on nanocrystalline anatase
Biological surface science and especially the interplay of titania surfaces and biomolecules are of great interest in several fields, such as biomineralization, where composite materials are formed, which have mechanical properties superior to the inorganics [1]. For medical implants in the human body, titanium is a very important inorganic implant material [2]. Single amino acids may determine the surface orientations of titania nanocrystals [3, 4]. Small peptides with 10 to 20 amino acids control nucleation [5] and structural formation of minerals and provide molecular scaffolds in formation of hard tissue [6].
In the field of biotechnology immobilization of biomolecules onto solid supports is mandatory to obtain a biologically functional material. The production of stable, functional and high surface density molecular films to improve in situ performance is strongly required for all biotechnological applications, ranging from biosensors to tissue engineering [7].
Titania TiO 2 is a wide band gap semiconductor, which can be synthesized on the nanometer scale exhibiting unique photoelectronic and photochemical properties [8]. It has been utilized for photodynamic therapy [9], in generating superhydrophilic surfaces [10], as well as in a new generation of transistors and biosensors [11, 12]. Functional biomolecular coatings such as proteins and peptides that are strongly and specifically adsorbed to the oxide may further advance the development of TiO 2 -based na-nobiotechnological applications.
The peptide affinity toward titania nanoparticle surfaces depends on the constituent amino acids, which are available for interaction. Therefore, studying the adsorption of individual amino acids on TiO 2 nanoparticle surfaces can provide valuable insights into the peptide affinity to TiO 2 nanoparticles [13].
Majority of reports describe amino acid interactions with titania surface in qualitative manner. That is due to the prevalence of spectroscopic and computer based methods in the sorption studies. Only two papers provide substantial data on thermodynamics, although it was obtained for different sorbates at surfaces with different crystallinity, which makes comparative analysis impossible [13, 14]. Despite the extensive utilization of the biomolecule – titania interfaces, interactions at the aqueous titania interface remain far from being fully understood. Without such an understanding, it will be challenging to design, predict, and/or optimize peptide adsorption and the concomitant properties of the resulting biointerface, amenable to utilization. Here we present the studies of bulk adsorption of major amino acids on nanocrystalline anatase at uniform conditions mimicking biological medium.
Experimental
Chemicals . Titanium isopropoxide, 2-( N -morpholino)ethanesulfonic acid (MES) and trifluoroacetic acid (TFA) were purchased from TCI Chemicals and used as received. n -Propanol, trimethylamine and phenyl isothiocianate were purchased from Sigma and used without purification. L -Amino acids, 99% grade, were purchased from Sigma. Acetonitrile, HPLC grade, was received from BioSolve. Deionized water was obtained on the spot.
Nanocrystalline anatase was prepared in accordance with previously established procedure [15]. Briefly, 22.5 mL of isopropanol was mixed with 2.5 mL of titanium(IV) isopropoxide and let mixing for 1 hour, after that 1.25 mL of deionized water was added and the mixture was heated under reflux condenser for 16 hours. The resulting slurry was centrifuged to separate the solution from the precipitate. The precipitate was dried in vacuum for 24 hours, followed by calcination at 400 °C, for 1 h.
Stock solutions and buffer. Stock solutions were prepared by dissolving dry amino acids in deionized water with concentrations of 2, 4, 8 and 16 mM. In the case of Tyrosine stock solutions were 0.5, 1 and 2 mM due to limited solubility of the amino acid. The MES buffer with the concentration of 10 mM was prepared from dry 2-( N -morpholino)ethanesulfonic acid; its pH was adjusted to 7.4 with dry sodium hydroxide.
Adsorption of amino acids on TiO 2 . The sol of anatase nanoparticles (2 mg/mL, 2 mL) in deionized water and MES-buffer (10 mM, pH 7.4, 1 mL) was added to a series of amino acid dilutions (8, 4 and 2 mM, 1 mL). Resulting series of samples were stored in a thermostat at 0, 10, 20, 30 and 40 °C for 24 h to achieve the adsorption equilibrium. After storage the samples were filtered through syringe filter (Vladfilter) 0.2 µm and used for analysis.
Derivatization and HPLC analysis. Prior to analysis the samples were derivatized with Edman’s reagent [16]. Briefly, 400 µL of a sample was mixed with 400 µL of triethylamine and phenyl isothiocyanate solution in acetonitrile (50 mM each). The mixture was thermostated at 60 °C for 15 min, neutralized with of 225 µL TFA. 20 µL of the resulting solution were analyzed by HPLC (Shimadzu, LC-20 Prominence) equipped with UV-Vis detector (λ = 254 nm). Analysis was carried out using reversed phase Zorbax column, 150X2.5 mm, 5 µm. Mobile phase consisted of 0.1 % TFA in deionized water and pure acetonitrile, with acetonitrile gradient from 20 % to 90 % in 13 min.
Fourier transform infrared spectroscopy (FTIR). The IR spectrum in the range 4000–400 cm–1 for a KBr pellet was recorded by a Shimadzu IRAffinity-1S FTIR spectrometer.
X-ray diffractogram was recorded by a RIGAKU ULTIMA IV device operating on Cu Kα radiation (λ=0,154 nm).
Specific surface area was measured by the means of a ASAP2020 Micromeritics device. The principle of measurement was the adsorption of N 2 at 77K. Total surface area was calculated based on BET method, at the pressure ranging from 0.1 to 0.3 P/P o .
Nuclear magnetic resonance spectra were recorded by a Varian-VNMRS spectrometer operating at 500 MHz in deuterium oxide solution using HDO-signal as a reference.
Quantum-chemical calculations . The calculation of the 1H NMR spectra of L -alanine was completed using GIAO [17] DFT B3LYP at 6-311G+(2d,p) level of theory within GAMESS software, release May 2013 R1 [18, 19]. The modeling was performed both for zwitterionic and for molecular (neutral) form of L -alanine. The conformational search and global minimum search was carried out using the above-mentioned level of theory.
Results and Discussion
Nanomaterial Characterization. The XRD pattern of the calcined sample is presented on Fig. 1a. The most intensive peak at 25 2 Ѳ degrees corresponds to (101) facet of the crystalline anatase phase of TiO 2 [15]. The rutile and brookite most intensive peaks at 27 and 30 2 Ѳ degrees respectively have not been detected, which means that the crystalline phase is 100 % anatase. Average crystal size has been calculated with Scherrer equation using FWHM (full width at half maximum) of the most intensive anatase peak at 25 2 Ѳ degrees and corresponds to 8.2 nm. The nitrogen adsorption isotherm is presented on Fig. 1b. Specific surface area has been calculated by BET method and found to be 131.9 m2/g.
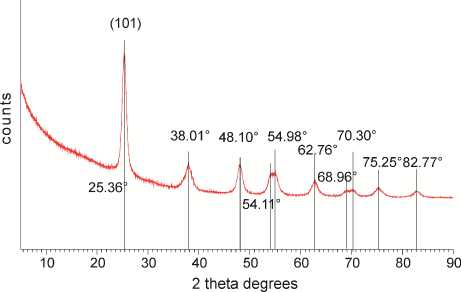
a)
Fig. 1. Characterization of nanocrystalline anatase: а) XRD pattern; b) N 2 sorption isotherm
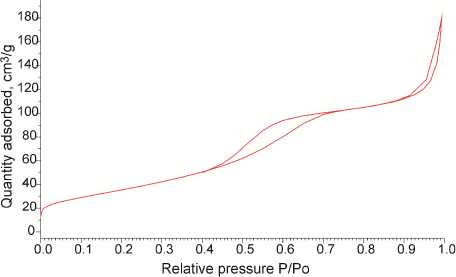
b)
The FTIR pattern of nanocrystalline anatase sample is depicted on Fig. 2. The widest and the most intensive band at 400–900 cm–1 is attributed to the titanium dioxide lattice vibrations. The adsorption band at 3000–3700 cm–1 corresponds to the surface hydroxyl group vibrations, while the small band at 1650 cm–1 corresponds to the O-H bonds of inner structure.
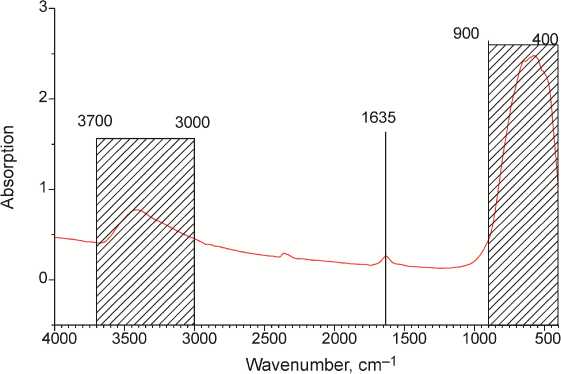
Fig. 2. The FTIR spectrum of nanocrystalline anatase
A dsorption of amino acids on nanocrystalline TiO 2. Adsorption studies of amino acids at uniform conditions require pH stability. The pH value strongly influences the surface charge and the charge of the sorbate itself. This is why we employed MES-buffer with pH 7.4, relating to physiological medium. We refrained from using conventional phosphate buffer as phosphate ions were known to suppress amino acid adsorption [14]. We have chosen the non-coordinating MES-buffer, as it is incapable of forming even weak complexes with metal ions, and thus do not coordinate surface titanium [20].
Adsorption isotherms of amino acids at different temperatures are given on Fig. 3.
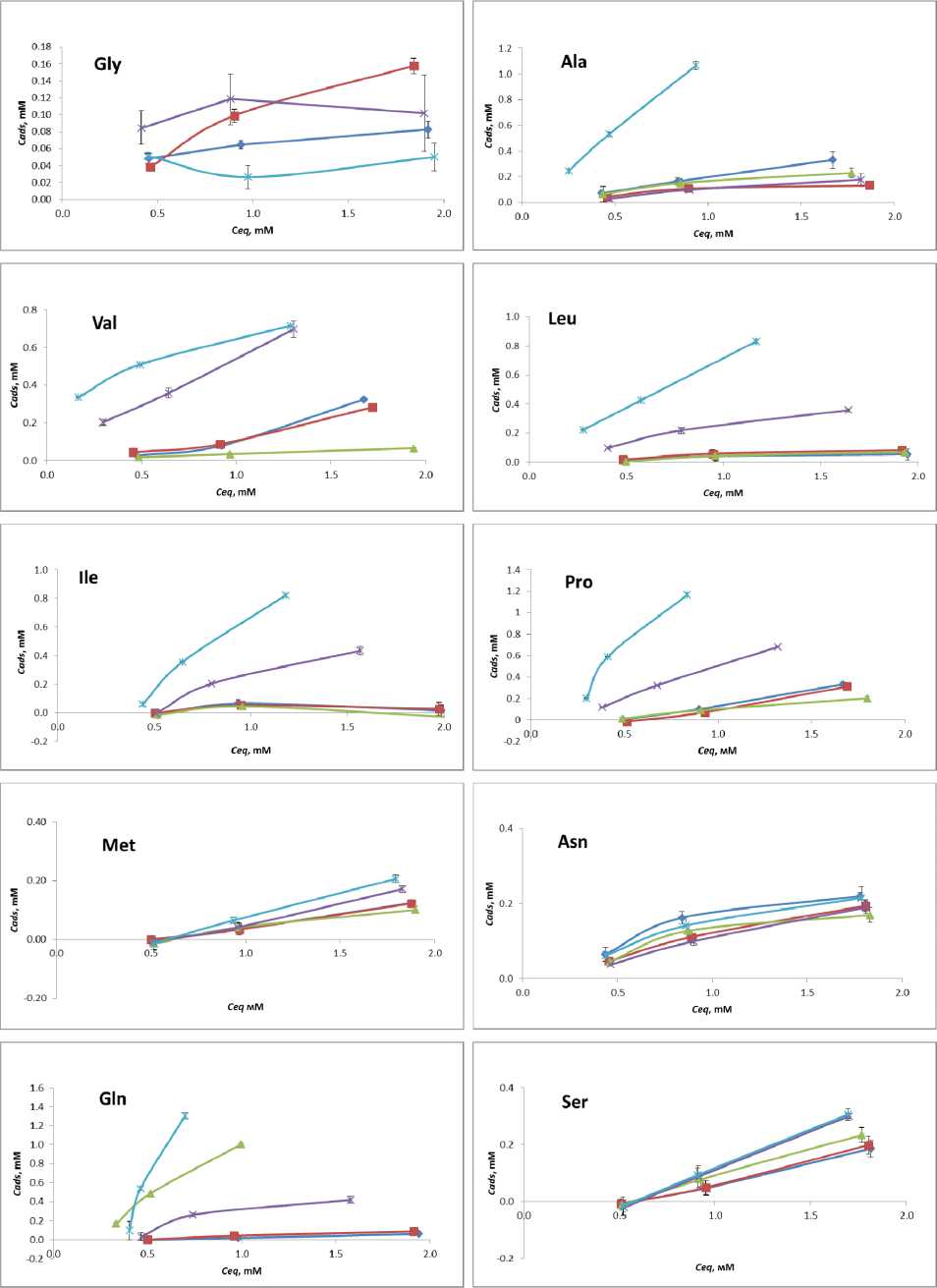
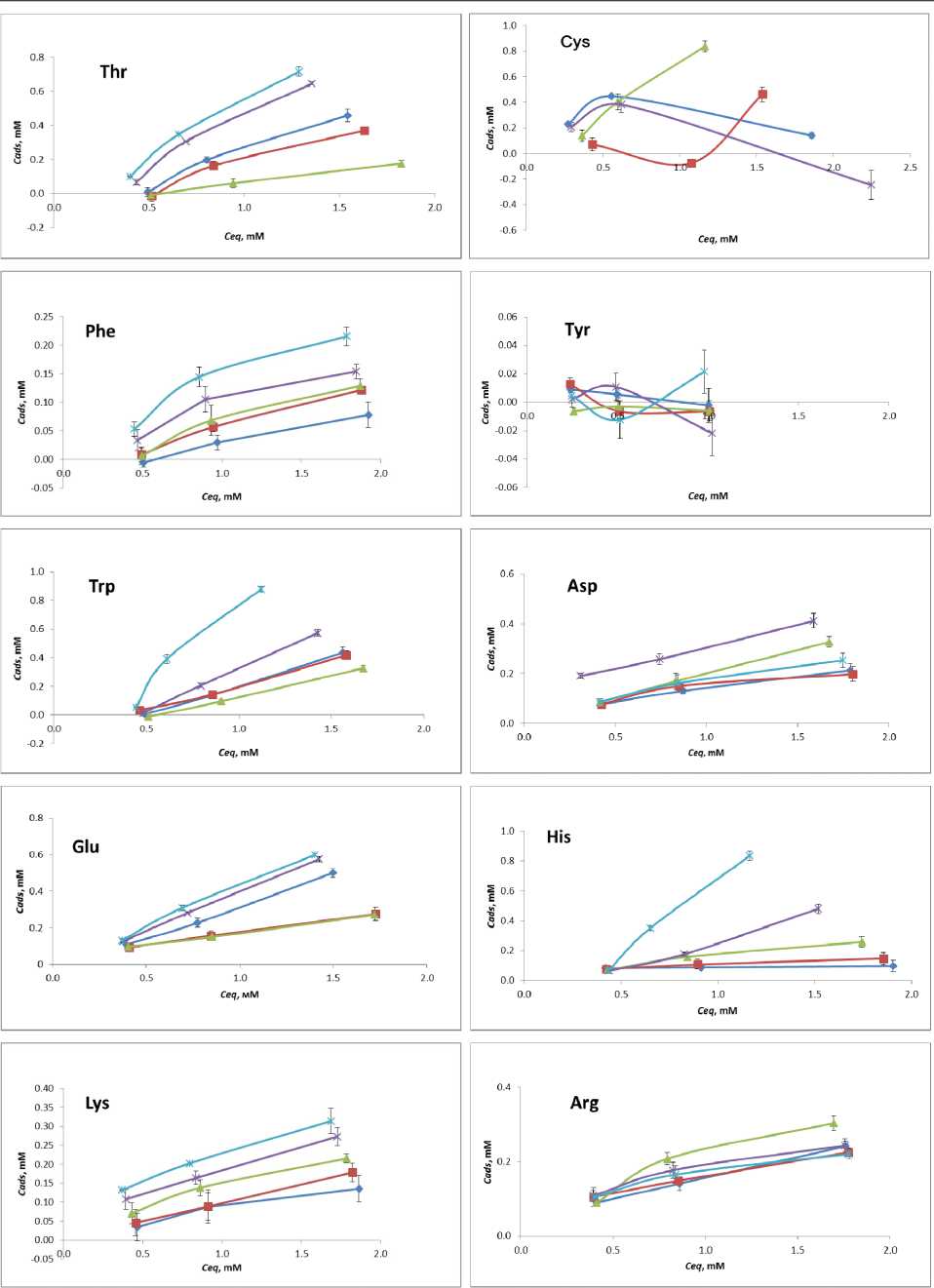
Fig. 3 L- Amino acid adsorption isotherms: -Ф-О°С-е-10°С 20 °C ^-30 °C -*-40 °C
Some of the isotherms fall into the Langmuir model of single monolayer saturation. Assuming monolayer adsorption of an amino acid on the TiO2 nanoparticle surface at pH 7.4 and it being reversible at 24 h, the experimental data can be fit using the Langmuir model, according to equation max
C _ KC Ads [ C eq ] ads” 1 + K [ Ceq ] ’
where Cads is the adsorbed amino acid, C eq is the unbound amino acid at equilibrium, C A m d a s x is the amino acid saturation, and K is the equilibrium binding constant. Other isotherms are represented as linear graphs, without saturation. Additional adsorption studies are required for elucidation of C A m d a s x and revelation of K.
The majority of amino acids show increased sorption with the temperature rise. Met, Phe, Ser and Lys show gradual increase in adsorption. Pro, Leu, Ile, Gln and His exhibit negligibly low adsorption at low temperatures, while at 30 to 40 °C they perform spike-like affinity increase. Glycine performs low or negligible adsorption at given temperatures at pH 7.4, which is in good compliance with the previous study [21]. Tyrosine shows similar behavior. As for Arg and Asp , at pH 7.4 no significant influence of temperature on their sorption has been noticed.
For amino acids Val, Ala, Trp, Thr, Glu we have observed the reverse of the adsorption trend within 0 to 40 °C temperature range. With gradual decrease of adsorption at low temperatures, the amino acids start adsorbing stronger on titania at 30 to 40 °C. To the best of our knowledge, current literature do not include any similar examples of such sorption behavior.
FTIR-studies. One of the possible explanations for increased sorption at elevated temperatures might be chemisorption of amino acids on titania. Only one example of amino acid chemisorption on titania is known, where homocysteine is chemisorbed at slightly acidic pH [22]. Chemisorption leads to new chemical bonding between a sorbate and the surface, which is stronger than electrostatic, hydrogen or Wan-der-Waals bonding at physisorption. In a way of proving chemisorption at elevated temperatures, we analyzed the IR-spectra of titania after adsorption of Ala at 40 °C, as IR-spectroscopy is the most convenient method for identification of newly formed bonds. In order to do that the titania sorbent was thoroughly rinsed with deionized water after sorption, dried at vacuum and analyzed with IR-spectroscopy. Joint spectrum of titania before and after adsorption is depicted on Fig. 4. The IR-spectrum of titania after Ala sorption at 10 °C is also included as the physisorption reference.
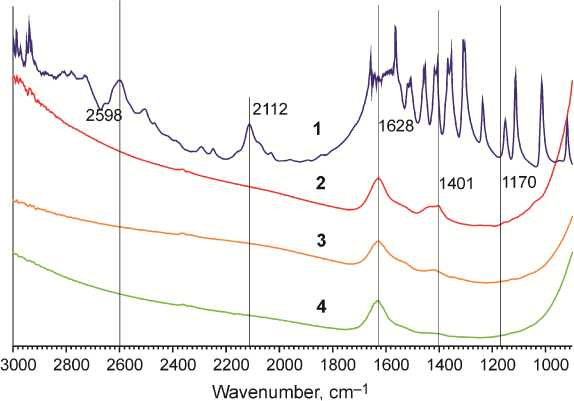
– L -Alanine
– dry pureTiO 2
– TiO 2 at 40 °C
– TiO 2 at 10 °C
Fig. 4. FTIR spectra of nanocrystalline anatase before and after L-Alanine
There are no substantial differences in the spectra of pure dry titania and titania samples obtained after adsorption of Ala at 40 °C. The same applies to the sample obtained after adsorption of Ala on titania at 10 °C. All of the three spectra have bands at 1628 and 1401 cm–1 corresponding to titania surface OH-groups. We expected formation of new Ti-O-C bonding between carboxylic residue and surface Ti-OH group, which would give a band around 1170 cm–1 of an IR-spectrum [23]. In case of chemisorption we would also expect to witness characteristic Ala bands at 2112 and 2598 cm–1. None of the abovementioned signs of chemisorption has been detected. Thus we conclude that increased sorption of Ala at high temperatures remains to be physisorption.
NMR studies. Stronger affinity of Ala towards nanocrystalline anatase at 30 and 40 °C could be explained by changes in molecular geometry of alanine, rather than crystalline changes of titanium oxide. Temperature-dependent geometry alterations of an amino acid molecule could be monitored with temperature resolved 1H NMR in deuterium oxide. Dynamics of chemical shifts corresponding to protons at α -carbon and side chains correlate with molecular geometry changing. We recorded the 1H NMR-spectrum of Ala 2 mM solution in D 2 O at 25, 30 and 40 °C. HDO signal was taken as an internal reference. Chemical shift of HDO at different temperatures has been set from equation:
5 ( HDO ) = 5.051 - 0.0Ш Т , (2) where T is the temperature in °C [24].
A typical 1H NMR spectrum of L -alanine in D 2 O is depicted in Fig. 5. The α -proton resonates at 3.7-3.8 ppm and methyl protons resonate at 1.46 ppm. The carboxylic and amine protons are masked in HDO signal due to strong proton exchange. Chemical shifts of L- alanine α -proton and methyl protons at different temperatures in D 2 O are summarized in Table 1.
It should be noted that the temperature value has little effect on chemical shifts. The difference between chemical shifts at 25 and 40 °C for the α- proton is only 0.008 ppm and for the methyl protons it equals 0.010 ppm. Both shifts decrease with temperature increase.
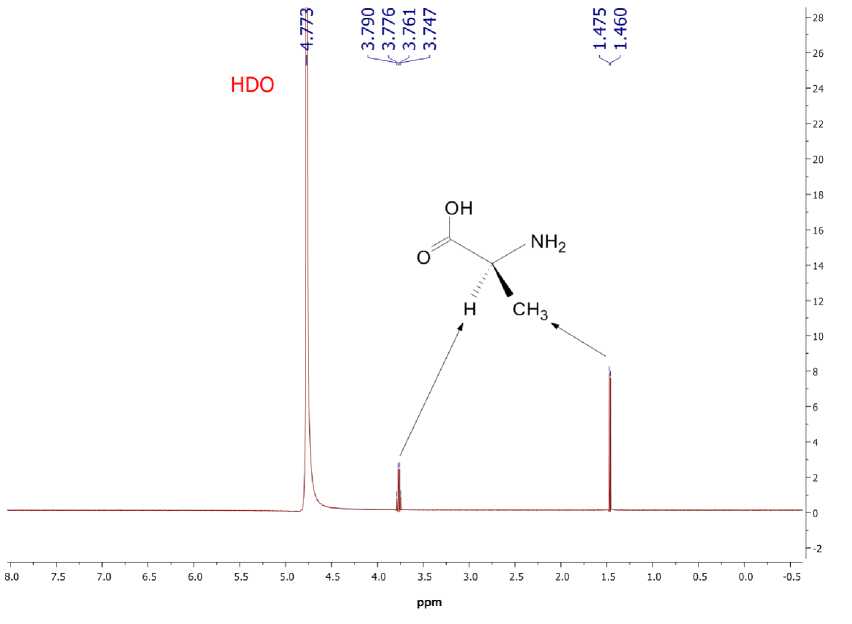
Fig. 5. The 1H NMR spectrum of L- alanine in D 2 O
Table 1
Characteristic 1H NMR signals of L- alanine in D 2 O
T , °C |
δ (HDO), ppm |
δ ( α -proton quadruplet), ppm |
δ (CH 3 doublet), ppm |
||||
25 |
4.773 |
3.790 |
3.776 |
3.761 |
3.747 |
1.475 |
1.460 |
30 |
4.718 |
3.789 |
3.775 |
3.760 |
3.746 |
1.473 |
1.458 |
40 |
4.607 |
3.782 |
3.768 |
3.753 |
3.739 |
1.465 |
1.450 |
The minima for zwitterionic and molecular (neutral) forms are represented in Fig. 6. Both forms have very similar structure involving N…H…O bundle but the location of H relatively to N and O is different. The calculated 1H NMR spectra for both forms are also similar to each other. The chemical shifts of L- alanine α -proton and methyl protons for zwitterionic and molecular (neutral) forms in D 2 O are summarized in Table 2. The calculated values of chemical shifts for both forms are mostly in agreement with the experimental ones. A small difference between chemical shifts of α -protons of zwitterionic and molecular (neutral) forms should be noted: the mean values of quadruplet differ by only 0.033 ppm and the quadruplet diapasons are intersected.
Table 2
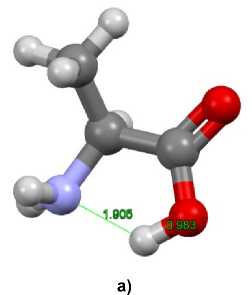
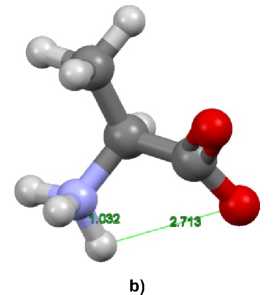
Characteristic 1H NMR signals for zwitterionic and molecular (neutral) forms of L-alanine in D 2 O
Tautomer |
δ ( α -proton quadruplet), ppm |
δ (CH 3 doublet), ppm |
||||
zwitterion |
3.579 |
3.521 |
3.511 |
3.465 |
1.663 |
1.650 |
molecular (neutral) form |
3.519 |
3.486 |
3.480 |
3.459 |
1.481 |
1.465 |
Fig. 6. Structures of alanine (a) molecular (neutral) and (b) zwitterionic forms
At the same time the chemical shifts of methyl protons of zwitterionic and molecular (neutral) forms differ significantly, the difference between their mean values for doublet is 0.183 ppm. The chemical shifts of α- proton and methyl protons for the molecular (neutral) form are less than for zwitterionic form. Comparing the experimental and computational results presented in Tables 1 and 2, it is possible to suppose an increase of the molecular (neutral) form concentration with temperature increase. It is quite natural as the zwitterion is the dominating form at room temperature. Obviously, the temperature increase usually yields gradual concentration equalization of all forms.
This behavior is determined by the chemical equilibrium theory. For the binary system “zwitterion –

(where Δ G is
molecule”, the fraction of the minor molecular form is proportional to pM = ex a difference of Gibbs free energies for the molecule and zwitterion, R is the gas constant, T is absolute temperature), while the fraction of the major zwitterion form is proportional to pZ = 1 - exp
A G ^
RT )
. In
agreement with the mean value theorem or the superposition principle, the chemical shift of the mixture of tautomers ( 5 ) can be determined as a sum of partial chemical shifts of each tautomer, taking into account their fractions, i.e.
5 = Z P i 8 i .
For the elucidated binary system, the sum in the equation involves only two terms:
5 = P m 5 Ni + P z 5 z , (4)
where δ M and δ Z are the chemical shifts of the molecular and zwitterionic forms of alanine, correspondingly. Substituting the p M and p Z by their values we can obtain the following equation
( A G
5 = 5 Ni exp l -— l + 5 Z
V RT 7
1 - exp
A G
-
RT
All the variables in the equation, excluding Gibbs free energy, are known for each studied temperature, which makes it possible to determine Δ G using nonlinear estimation techniques. The Δ G value determined using chemical shifts of methyl protons at all temperatures is 2.75 ± 0.12 kJ/mol. The Δ G value determined using chemical shifts of α- proton at all temperatures is 2.51 ± 0.18 kJ/mol. These values are in good agreement with the experimental data represented in [25], where the estimated value of Gibbs free energy for zwitterionic – molecular form transformation lies in the range 2 ÷ 3 kJ/mol depending on the concentration of the amino acid. The knowledge of the Gibbs free energy value allows estimating the portions of zwitterionic (Z) and molecular (M) form at each temperature. They are 0.671(Z) and 0.329(M) at 25 °C; 0.665(Z) and 0.335(M) at 30 °C; 0.653(Z) and 0.347(M) at 40 °C. The fraction of the zwitterionic form naturally decreases while the fraction of the molecular form naturally increases. The curves for both of the forms have an inflection point at 35 ± 2 °C. After this point the fraction of the molecular form increases dramatically, this can lead to the change of the sorption mechanism as noted above. It is interesting that the value of inflection point lays closely to the physiological temperature of most warm-blooded organisms, which can give a key for understanding of some biological phenomena.
Conclusions
Adsorption of native amino acids on nanocrystalline titania has been accomplished at the uniform conditions relating to those in the living organisms. Some of the established isotherms reveal monomo-lecular saturation adsorption of amino acids in accordance with the Langmur model. The majority of amino acids exhibits increased adsorption with the temperature rise. Pro, Leu, Ile, Gln and His exhibit negligibly low adsorption at low temperatures, while at 30 to 40 °C they perform spike-like affinity increase.
For amino acids Val, Ala, Trp, Thr, Glu we have observed the reverse of the adsorption trend within 0 to 40 °C temperature range. With gradual decrease of adsorption at low temperatures the amino acids start adsorbing stronger on titania at 30 to 40 °C. IR-spectroscopy has shown the absence of newly formed chemical bonds with titania at elevated temperatures thus rejecting hypothesis of chemisorption.
Quantum chemical calculations of Ala at the temperatures of 25 to 40 °C are in good agreement with temperature resolved 1H NMR. Increased adsorption of Ala at 40 °C corresponds to the equilibrium shift towards the molecular form of the amino acid.
Acknowledgements
The reported study was funded by RFBR according to the research project № 15-03-07834-a.
Список литературы Features of L-amino acid adsorption on nanocrystalline anatase
- Weiner S., Dove P.M. Biomineralization, Reviews in Mineralogy and Geochemistry, 2003, vol. 54, pp. 1-29.
- Albrektsson T., Branemark P.I., Hansson H.A., Kasemo B., Larsson K., Lundstrom I., McQueen D.H., Skalak R. The Interface Zone of Inorganic Implants In vivo: Titanium Implants in Bone. Annals of Biomedical Engineering, 1983, vol. 11, pp. 1-66 DOI: 10.1007/BF02363944
- Duruphthy O., Bill J., Aldinger F. Bioinspired Synthesis of Crystalline TiO2: Effect of Amino Acids on Nanoparticles Structure and Shape. Crystal Growth and Design, 2007, vol. 7, pp. 2696-2704 DOI: 10.1021/cg060405g
- Köppen S., Bronkalla O., Langel W. Adsorption Configurations and Energies of Amino Acids on Anatase and Rutile Surfaces. Journal of Physical Chemistry B, 2008, vol. 112, pp. 13600-13606 DOI: 10.1021/jp803354z
- Addadi L., Weiner S. Interactions Between Acidic Proteins and Crystals: Stereochemical Requirements in Biomineralization. Proceedings of National Academy of Science U.S.A., 1985, vol. 82, pp. 4110-4114 DOI: 10.1073/pnas.82.12.4110
- Oren E.E., Tamerler C., Sahin D., Hnilova M., Seker U.O.S., Sarikaya M., Samudrala R. A Novel Knowledge-Based Approach to Design Inorganic-Binding Peptides. Bioinformatics, 2007, vol. 23, pp. 2816-2822 DOI: 10.1093/bioinformatics/btm436
- Rezania A., Johnson R., Lefkow A.R., Healy K.E. Bioactivation of Metal Oxide Surfaces. Surface Characterization and Cell Response. Langmuir, 1999, vol. 15, pp. 6931-6939 DOI: 10.1021/la990024n
- Gratzel M. Photoelectrochemical cells. Nature, 2001, vol. 414, pp. 338-344 DOI: 10.1038/35104607
- Kubota Y., Shuin T., Kawasaki C., Hosaka M., Kitamura H., Cai R., Sakai H., Hashimoto K., Fujishima A. Photokilling of T-24 Human Bladder Cancer Cells with Titanium Dioxide. British Journal of Cancer, 1994, vol. 70, pp. 1107-1111 DOI: 10.1038/bjc.1994.456
- Sun R.D., Nakajima A., Fujishima A., Watanabe T., Hashimoto K. Photoinduced Surface Wettability Conversion of ZnO and TiO2 Thin Films. Journal of Physical Chemistry B, 2001, vol. 105, pp. 1984-1990 DOI: 10.1021/jp002525j
- Diebold U. Surface Science of Titanium Dioxide. Surface Science Reports, 2003, vol. 48, pp. 53-229 DOI: 10.1016/S0167-5729(02)00100-0
- Topoglidis E., Lutz T., Willis R.L., Barnett C.J., Cass A.E.G., Durrant J.R. Protein Adsorption on Nanoporous TiO2 Films: a Novel Approach to Studying Photoinduced Protein/Electrode Transfer Reactions. Faraday Discussions, 2000, vol. 116, pp. 35-46 DOI: 10.1039/B003313H
- Mudunkotuwa I.A., Grassian V.H. Histidine Adsorption on Nanoparticles: An Integrated Spectroscopic, Thermodynamic, and Molecular-Based Approach toward Understanding Nano-Bio Interactions. Langmuir 2014, vol. 30, 8751-8760 DOI: 10.1021/la500722n
- Okazaki S., Aoki T., Tani K. The Adsorption of Basic α-Amino Acids in an Aqueous Solution by Titanium(IV) Oxide. Bulletin of Chemical Society of Japan, 1981, vol. 54, pp. 1595-1599 DOI: 10.1246/bcsj.54.1595
- Praveen P., Viruthagiri G., Mugundan S., Shanmugam N. Structural, Optical and Morphological Analyses of Pristine Titanium Dioxide Nanoparticles -Synthesized via Sol-Gel Route. Spectrochimica Acta Part A: Molecular and Biomolecular Spectroscopy, 2014, vol. 117, pp. 622-629 DOI: 10.1016/j.saa.2013.09.037
- Heinrikson R.L., Meredith S.C. Amino Acid analysis by Reverse-phase High-Performance Liquid Chromatography: Precolumn Derivatization with Phenylisothiocyanate. Analytical Biochemistry, 1984, vol. 136, pp. 65-74 DOI: 10.1016/0003-2697(84)90307-5
- Cheeseman J.R., Trucks G.W., Keith T.A., Frisch M.J. A Comparison of Models for Calculating Nuclear Magnetic Resonance Shielding Tensors. J. Chem. Phys., 1996, vol. 104, pp. 5497-5509 DOI: 10.1063/1.471789
- Schmidt M.W., Baldridge K.K., Boatz J.A., Elbert S.T., Gordon M.S., Jensen J.H., Koseki S., Matsunaga N., Nguyen K.A., Su S.J., Windus T.L., Dupuis M., Montgomery J.A. General Atomic and Molecular Electronic Structure System. J. Comput. Chem., 1993, vol. 14, pp. 1347-1363 DOI: 10.1002/jcc.540141112
- Gordon M.S., Schmidt M.W. Advances in Electronic Structure Theory: GAMESS a Decade Later. Chapter 41. in: Theory and Applications of Computational Chemistry, the First Forty Years (C.E. Dykstra, G. Frenking, K.S. Kim, G.E. Scuseria, eds.). Amsterdam: Elsevier, 2005, pp. 1167-1189.
- Kandegedara A., Rorabacher D.B. Noncomplexing Tertiary Amines as "Better" Buffers Covering the Range of pH 3-11. Temperature Dependence of Their Acid Dissociation Constants. Analytical Chemistry, 1999, vol. 71, pp. 3140-3144 DOI: 10.1021/ac9902594
- Ojamae L., Aulin C., Pedersen H., Kall P.O. IR and Quantum-Chemical Studies of Carboxylic Acid and Glycine Adsorption on Rutile TiO2 Nanoparticles. Journal of Colloid Interface Science, 2006, vol. 296, pp. 71-78 DOI: 10.1016/j.jcis.2005.08.037
- Schmidt M.; Steinemann S. G. XPS Studies of Amino-Acids Adsorbed on Titanium Dioxide Surfaces. Fresenius’ Journal of Analytical Chemistry, 1991, vol. 341, pp. 412-415 DOI: 10.1007/BF00321947
- Chen J., Franking R., Ruther R.E., Tan Y., He X., Hogendoorn S.R., Hamers R.J. Formation of Molecular Monolayers on TiO2 Surfaces: A Surface Analogue of the Williamson Ether Synthesis. Langmuir, 2011, vol. 27, pp. 6879-6889 DOI: 10.1021/la2008528
- Gottlieb H.E., Kotlyar V., Nudelman A. NMR Chemical Shifts of Common Laboratory Solvents as Trace Impurities. Journal of Organic Chemistry, 1997, vol. 62, pp. 7512-7515 DOI: 10.1021/jo971176v
- Reichardt C., Welton T. Solvents and Solvent Effects in Organic Chemistry. 4th Edition. Berlin: Wiley-VCH, 2010. 718 p DOI: 10.1002/9783527632220